Biophysical Chemistry
Unveiling the Intersection of Physics, Chemistry, and Biology
Advancing Medicine Through Biophysical Chemistry
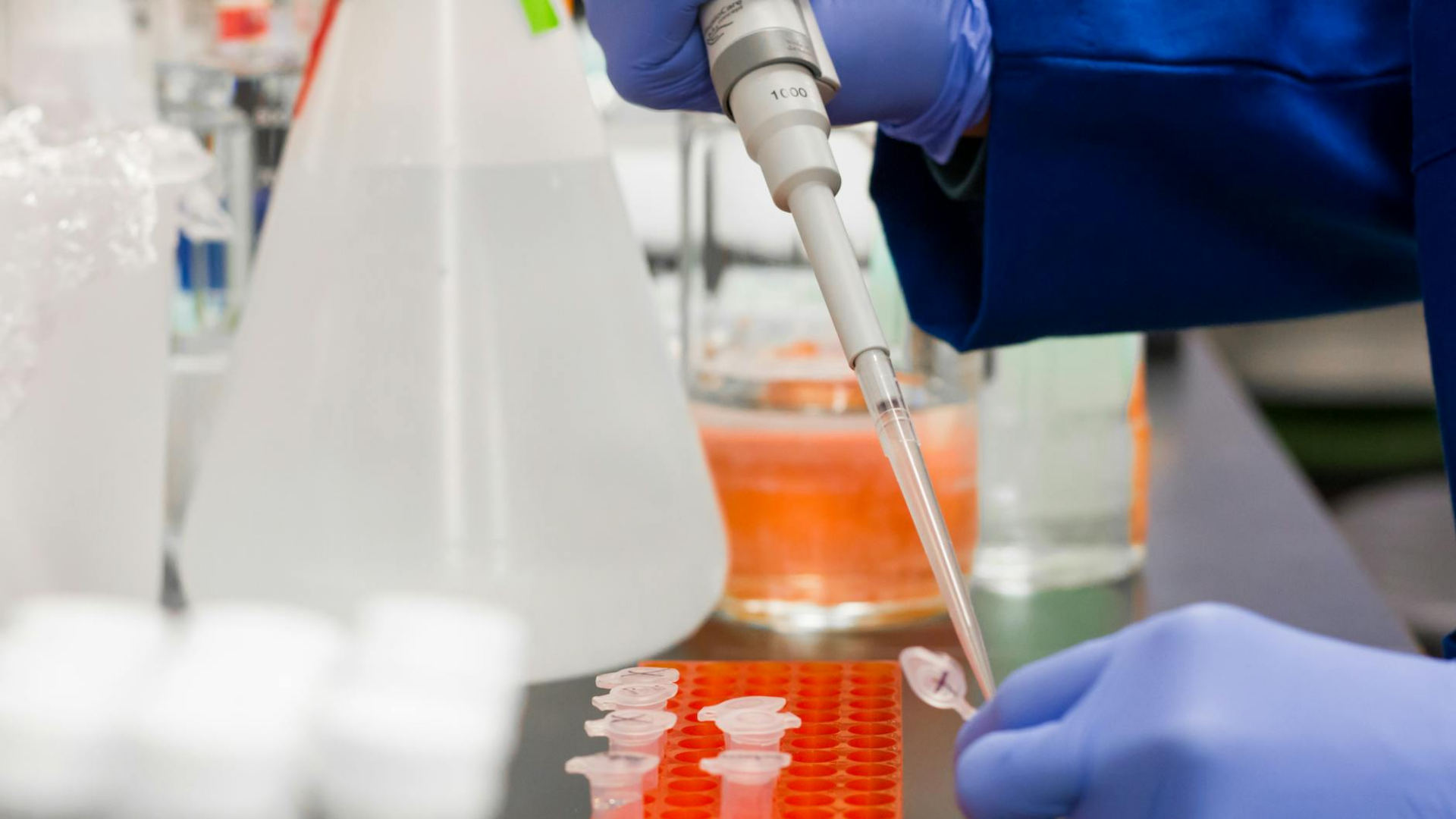
Biophysical chemistry is an interdisciplinary field that applies the principles and techniques of physics and chemistry to understand biological systems at a molecular level. It focuses on understanding the physical principles underlying the structure, function, and behavior of biological molecules, such as proteins, nucleic acids, lipids, and carbohydrates.
Biophysical chemistry applications span across scientific research, medical diagnostics, drug development, and pharmaceutical manufacturing. By providing detailed molecular-level information, biophysical chemistry plays a crucial role in advancing our understanding of biological processes and developing new therapeutic strategies.
From Prediction to Cure: Exploring Cancer Cells and Drug Responses
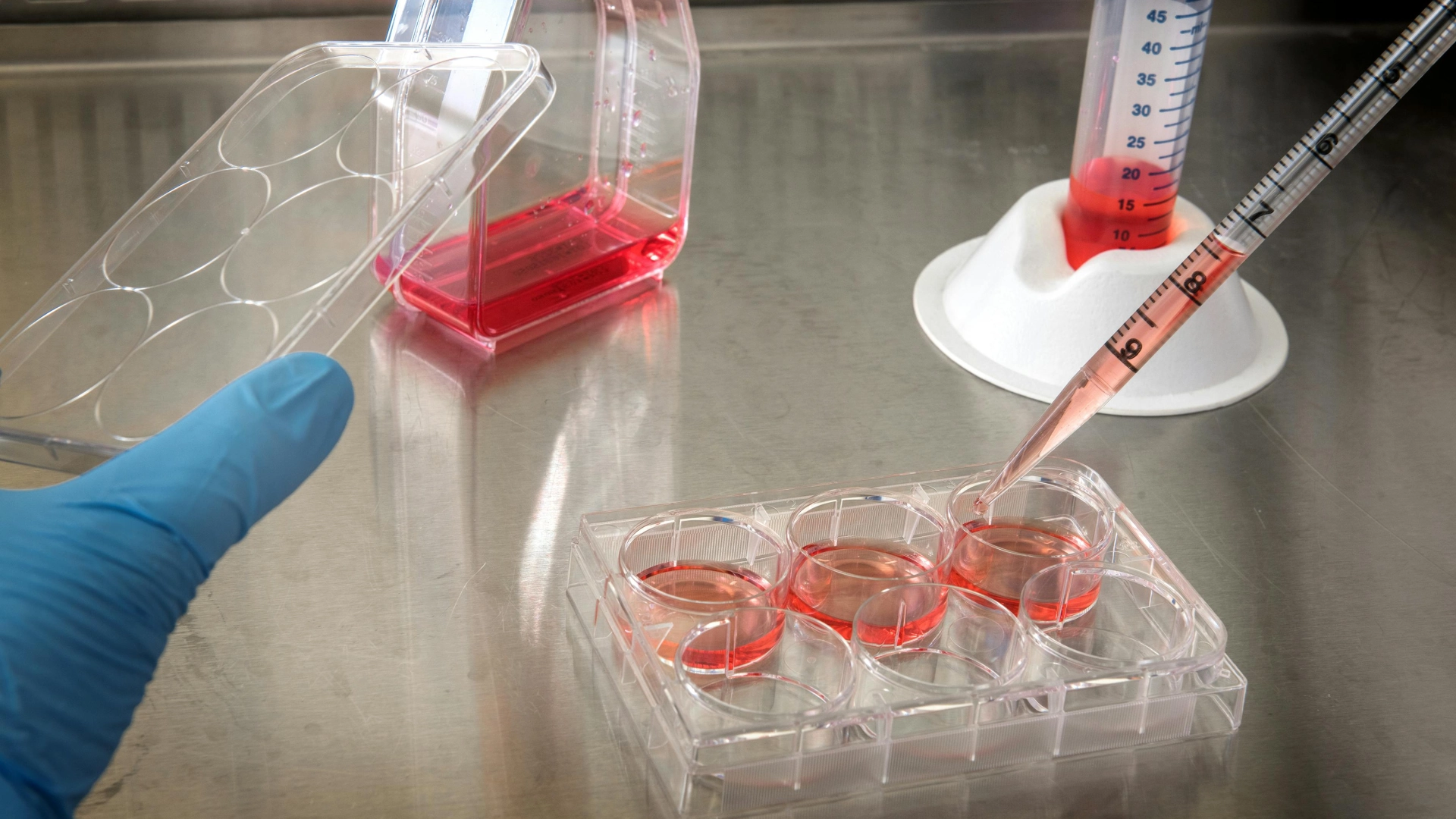
Human cancer cell lines are derived and isolated from real human tumors and are able to be grown and tested within the lab for a sufficient period of time. Scientists use them to study how cells behave and proliferate, how diseases develop, and how drugs work. These isolated human cancer cell lines are derived from various tumors, thus enabling scientists to study different types of cancer originating from different organs.
Cancer cells produce specific proteins that are crucial for their growth and proliferation. Computational (in silico) methods help predict how compounds interact with these proteins, but experimental validation is necessary. In vitro studies involve testing compounds on isolated proteins or cancer cells to observe their effects on growth, proliferation, and gene expression. In vivo studies use animal models to assess the overall biological impact.
Our research group investigates these compounds in human cancer cells with varying protein expression levels to uncover their mechanisms of action. One of our projects is dedicated to breast and lung cancer, utilizing in vitro studies with cancer cell lines to:
- verify computer-generated predictions by comparing them with real cell responses.
- test the effects of natural and novel compounds on cancer growth.
- study how mutations influence cancer progression.
- explore drug combinations and repurpose existing medications for better treatments.
By combining in silico, in vitro, and in vivo methods, we aim to identify promising cancer therapies.
Cancer Metabolism
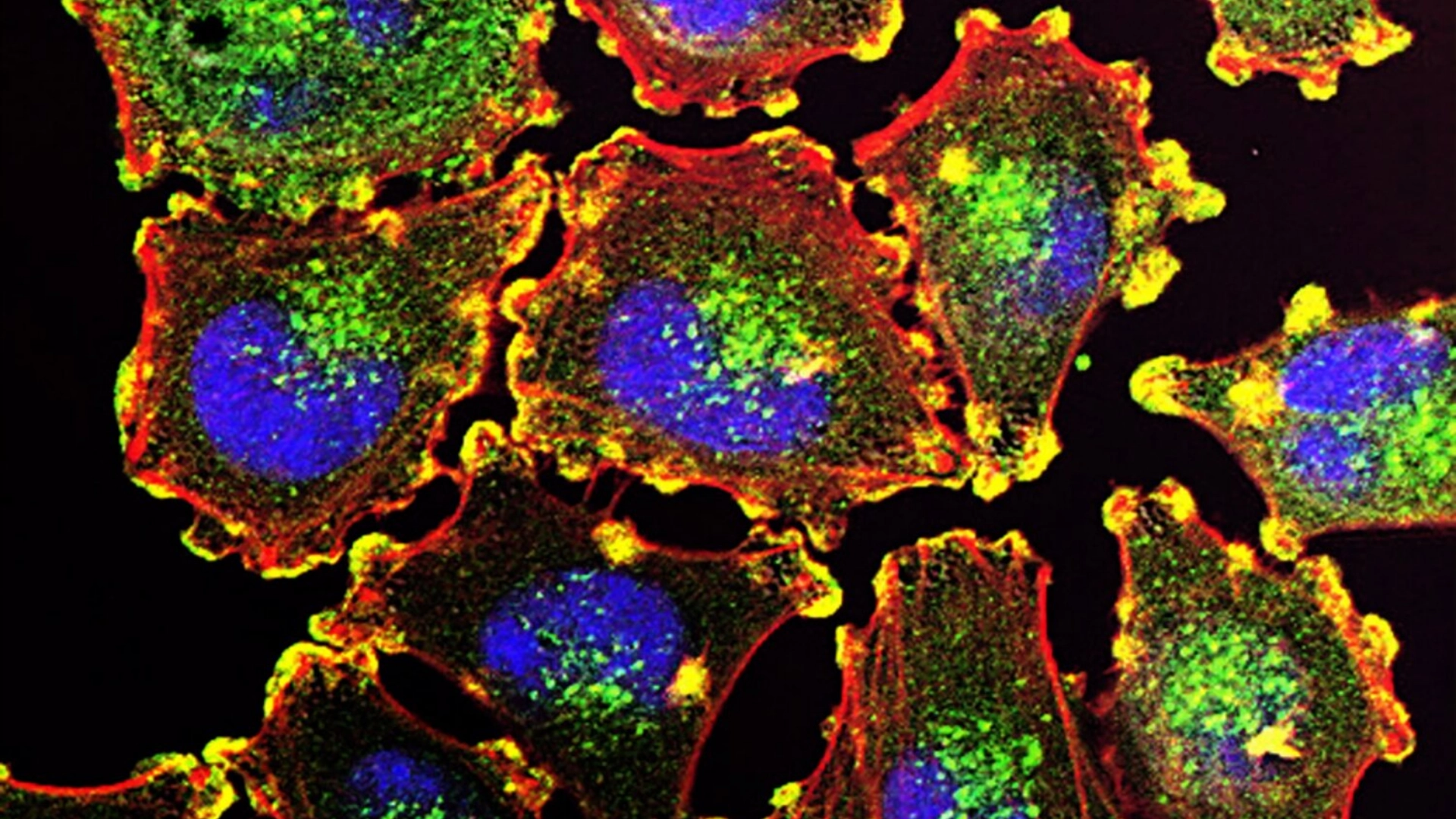
Bioluminescence
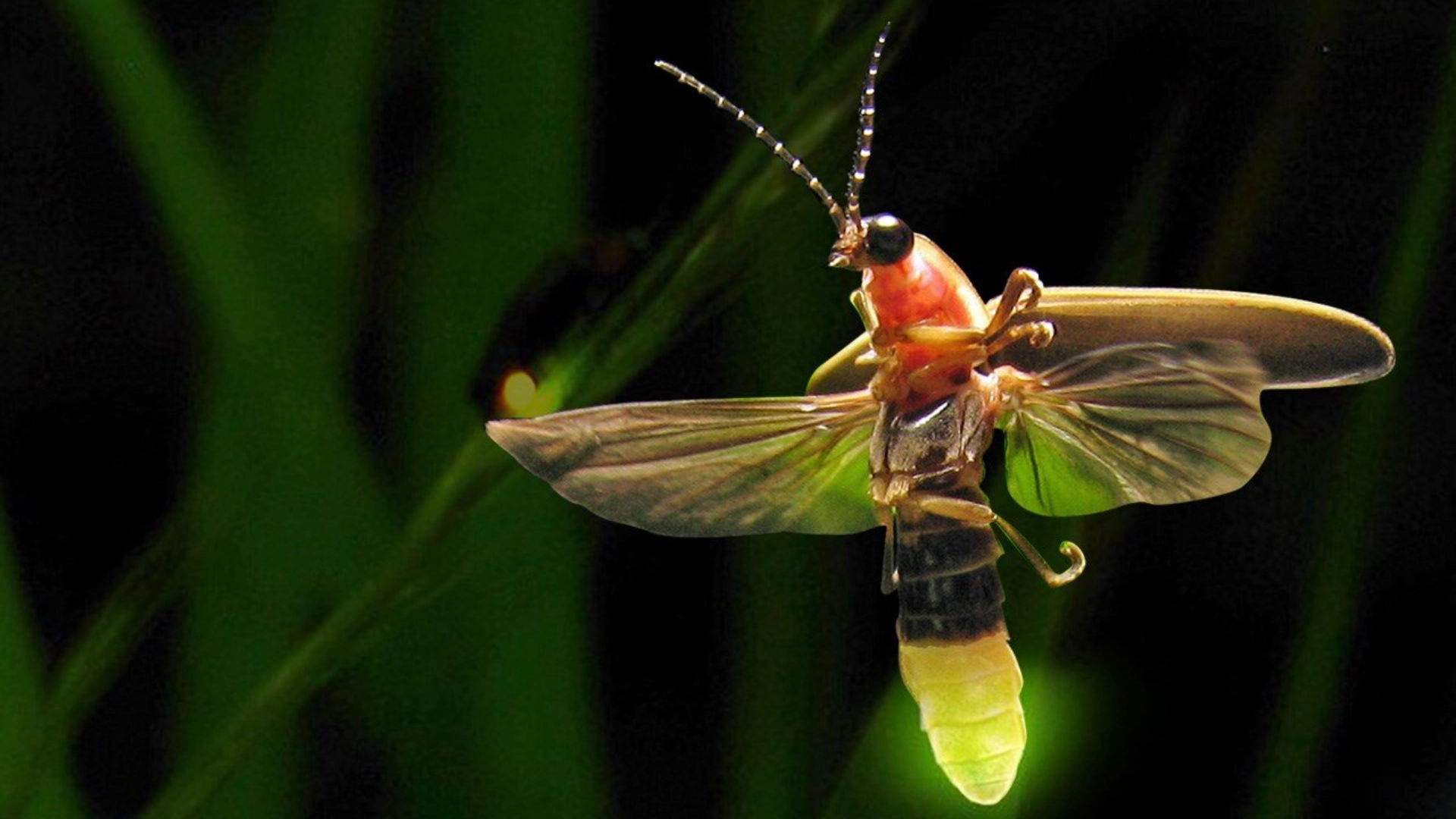
The Fascinating Bioluminescence of Fireflies:
The bioluminescent light emitted by fireflies has captivated scientists for centuries, sparking curiosity about how they produce light in the dark. This natural phenomenon involves a chemical reaction that generates light without heat, known as “cold light.” The unique ability of fireflies to emit different colors of light from the same reaction has been particularly intriguing. This variation in color emission, ranging from green to yellow to red, is not random but is influenced by several factors, including the molecular structure of the luciferin substrate and the presence of specific enzymes.
The Role of Luciferase Enzymes in Light Emission:
At the heart of firefly bioluminescence are enzymes called luciferases. These enzymes catalyze the reaction that converts chemical energy from the substrate luciferin into light. When luciferin interacts with oxygen in the presence of luciferase, it undergoes an oxidation reaction, producing oxyluciferin and emitting light in the process. The color of the emitted light is determined by the energy transition of oxyluciferin. Different luciferase variants and environmental conditions, such as pH and temperature, can influence this energy transition, resulting in the production of different wavelengths (colors) of light.
Applications of Bioluminescence in Science and Technology:
Understanding the mechanisms behind the production of various light colors has broad applications in science and technology. For instance, bioluminescence is increasingly used in biomedical research, including in vivo imaging to track disease progression or monitor cell proliferation. It also plays a key role in studying protein folding and secretion, as well as understanding cellular dynamics. Beyond the lab, bioluminescent reactions are useful in environmental monitoring and food quality control, offering non-invasive, real-time detection methods. By exploring the intricacies of this natural light production, scientists can harness it for innovations across a range of fields.
COVID-19
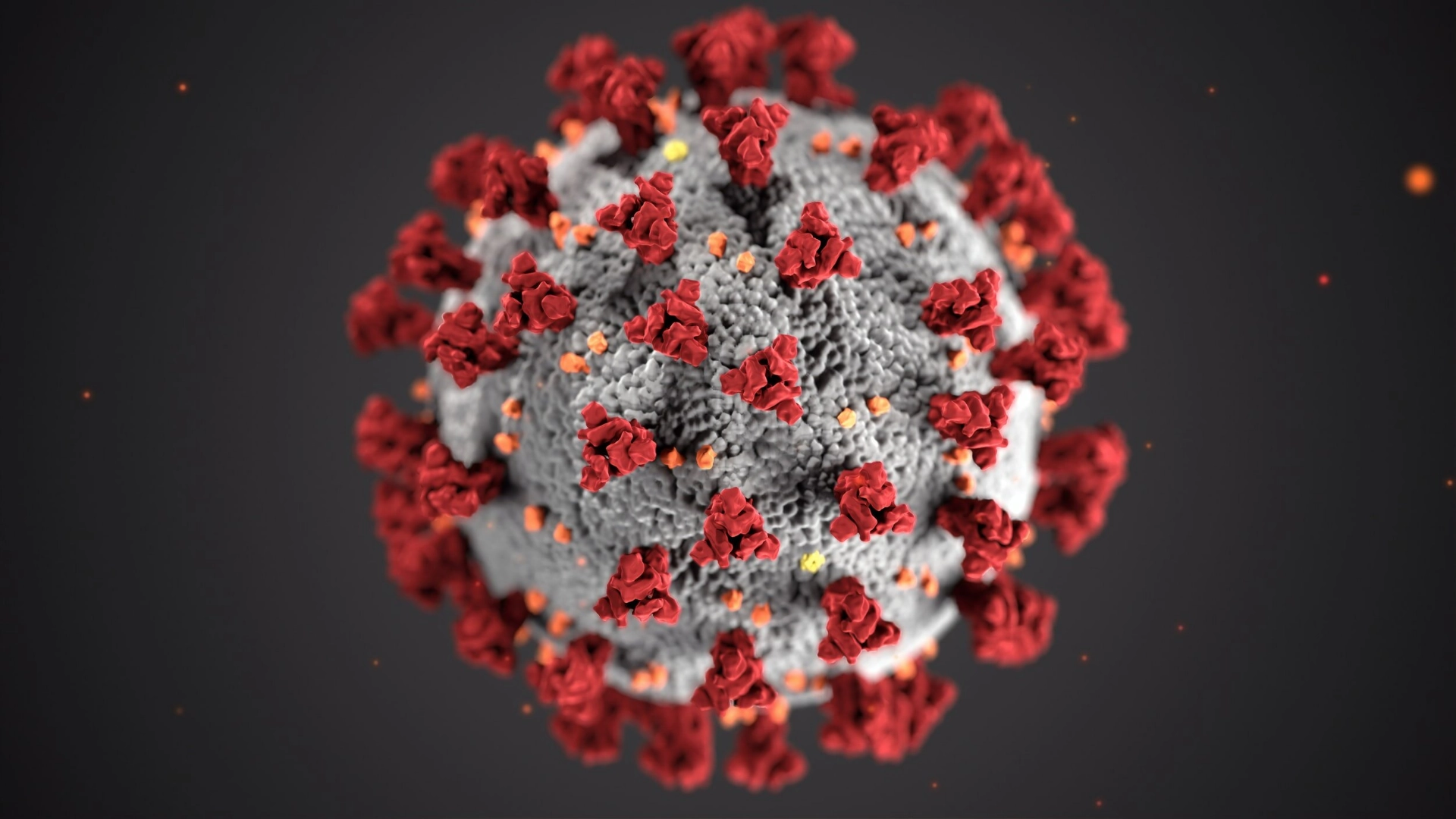
The Global Impact of COVID-19:
COVID-19, caused by the SARS-CoV-2 virus, has rapidly spread worldwide, leading to a global health crisis. The pandemic has overwhelmed healthcare systems, led to millions of deaths, and significantly disrupted economies and daily life. The virus’s highly contagious nature, combined with its ability to mutate into new variants, has made controlling its spread a challenge. Governments, scientists, and healthcare workers have been working relentlessly to develop strategies, treatments, and vaccines to mitigate the impact of the disease. However, the need for effective therapeutic solutions remains crucial in curbing the pandemic.
Targeting SARS-CoV-2 Proteases for Therapeutic Development:
One promising approach to controlling the spread of SARS-CoV-2 is the discovery of therapeutics that can inhibit the progression of the virus. The key to this strategy lies in targeting the viral proteases, specifically 3CLpro (Main protease) and PLpro (Papain-like protease), which are essential for the virus’s life cycle. These proteases help process the polyproteins produced during viral replication, releasing functional proteins necessary for assembling new virus particles. By inhibiting these enzymes, we can block the production of viral proteins, thereby halting the virus’s ability to replicate and spread within the host.
Characterizing Proteases for Antiviral Drug Discovery:
Our lab is focused on biochemically and biophysically characterizing the 3CLpro and PLpro proteases to support the discovery and development of antiviral therapeutics. Through detailed studies of their structure and function, we aim to identify potential drug candidates that can effectively inhibit these proteases. This research is crucial because understanding how these enzymes work at a molecular level allows us to design targeted inhibitors that can block their action without affecting the host cells. The ultimate goal is to develop antiviral treatments that can reduce the viral load, limit disease progression, and contribute to controlling the COVID-19 pandemic.